Applied Spectroscopy
The Laser Diagnostics group headed by Prof. Thomas Dreier and Dr. Torsten Endres is devoted to the development and practical application of optical measurement technique for use in combustion systems or other reactive flows. This includes laser-based methods such as Rayleigh scattering, spontaneous Raman scattering, laser-induced fluorescence (LIF), laser-induced incandescence (LII), and diode-laser absorption spectroscopy.
The team carries out fundamental experiments that enable the quantitative interpretation of measurements even in complex situations. This includes the determination of fluorescence lifetimes of small molecules (OH radical, formaldehyde, toluene, naphthalene) to clarify intra- and intermolecular energy transfer processes that are responsible for variations in signal intensity and spectra. Another example is the identification of light absorption in thin films of aqueous solutions in the near-infrared spectral range. In addition, the group also contributes to the development of laser-optical techniques (such as LII) used to measure the size of gas-borne nanoparticles – e.g. for measuring soot in engines or nanoparticles in synthesis reactors.
The measurement techniques are employed for identifying important parameters in flames or other environments that are of interest from a practical point of view. One focus is on imaging techniques for two-dimensional visualization of mixing, reaction, and flow. The thickness and temperature of aqueous liquid films is measured using tunable NIR diode lasers via absorption spectroscopy for the time-resolved analysis of quickly evaporating liquid films on hot surfaces. Contact-free quantitative measurement of heat release in turbulent flames are carried out with LIF imaging of OH and formaldehyde as well as time- and spectrally-resolved detection of chemiluminescence (OH*, CH*, CO2*).
Sooting Flames
Spectroscopy in sooting flames
Laser-Induced Incandescence (LII)
Several laser-based techniques offer the possibility of soot diagnostic in combustion processes. They allow the assessment of soot concentration, soot particle size distribution, soot structure etc. Laser-induced incandescence (LII) allows particle size measurements and two-dimensional imaging of soot properties. The same strategy can be used for diagnostics on particles of a wide variation of chemical composition. LII, therefore, is not only used for combustion diagnostics but is also applied in material synthesis of nano particles in the gas phase.
Following Planck´s law, hot particles emit thermal radiation. This causes the orange color of sooting (e.g. candle) flames. The particles in these flames can reach temperatures of up to 2000 K. In the laser-induced incandescence technique, the soot particles are additionally heated by an intense laser beam to their vaporization temperature of approx. 4000 K [1]. The heated particles show an emission characteristic ("incandescence") that is strongly different from the non-heated particles (more intense radiation, blue-shifted emission maximum, different temporal emission characteristics). This allows selective detection of the incandescence with fast detectors and image-intensified cameras.
Theoretical considerations [2] and experiments [3] have shown that the LII signal is directly proportional to soot volume fraction. LII, therefore, can give two-dimensional images of the soot concentration distribution in a flame [4].
Moreover, time-resolved measurements provide access to soot paricle size (time-resolved LII, TR-LII) [5]. After laser heating, the particles slowly cool down again until they reach ambient flame temperature some 500 ns after the laser pulse. During this cooling process they change their emission characteristics. Since large particles cool down slower than smaller ones, time-resolved emission measurements after excitation allow the determination of soot particle size distributions.
Das in der Arbeitsgruppe entwickelte Simulationprogram LIISim (www.liisim.com) ist in der Lage, den Verlauf von LII-Signalen vorherzusagen und gemessene Signalverläufe zu evaluieren.
LIISim - Software
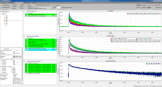
LIISim is a modular toolbox for signal processing and data analysis of laser-induced incandescence measurements. The open-source software was developed in our group for systematic data analysis and comparison.
Physical properties of investigated particles can be defined in database-files and allow evaluation of LII signal traces for various material systems (soot, silicon, germanium,…).
Detailed information, download links and documentation: http://www.liisim.com
References
[1] B. F. Kock, T. Eckhardt, and P. Roth, "In-cylinder sizing of Diesel particles by time-resolved laser-induced incandescence (TR-LII)," Proc. Combust. Inst. 29, 2775-2781 (2002).
[2] M. Hofmann, W. G. Bessler, J. Gronki, C. Schulz, and H. Jander, "Investigations on laser-induced incandescence (LII) for soot diagnostics at high-pressure," in Laser Applications to chemical and environmental analsyis, OSA Technical Digest Series (Optical Society of America, Washington DC, 2002), p. FC1/1-FC1/3.
[3] M. Hofmann, W. G. Bessler, C. Schulz, and H. Jander, "Laser-induced incandescence (LII) for soot diagnostics at high pressure," Appl. Opt. 42, 2052-2062 (2003).
[4] C. Schulz, B. F. Kock, M. Hofmann, H. A. Michelsen, S. Will, B. Bougie, R. Suntz, and G. J. Smallwood, "Laser-induced incandescence: recent trends and current questions," Appl. Phys. B, DOI: 10.1007/s00340-006-2260-8 (2006).
[5] A. Eremin, E. V. Gurentsov, M. Hofmann, B. F. Kock, and C. Schulz, "Nanoparticle formation from supersaturated carbon vapor generated by laser photolysis of carbon suboxide," J. Phys. D., DOI: 10.1007/s00340-006-2199-9 (2006).
[6] B. F. Kock, C. Schulz, and P. Roth, "Time-resolved LII applied to soot particle sizing and concentration measurements in the cylinder of a Diesel engine," Combust. Flame, in press (2006).
[7] A. V. Filippov, M. W. Markus, and P. Roth, "In situ characterization of ultrafine particles by laser-induced incandescence: Sizing and particle structure determination," Journal of Aerosol Science 30, 71-87 (1999).
[8] B. F. Kock and P. Roth, "Two-color TR-LII applied to in-cylinder Diesel particle sizing," in Proc. of the European Combustion Meeting (Orléans, 2003).
[9] B. F. Kock, C. Kayan, J. Knipping, H. R. Orthner, and P. Roth, "Comparison of LII and TEM sizing during synthesis of iron particle chains," Proc. Combust. Inst. 30, 1689-1697 (2005).
[10] B. F. Kock, C. Kayan, J. Knipping, H. R. Orthner, and P. Roth, "Comparison of LII and TEM sizing during synthesis of iron particle chains," Proceedings of the Combustion Institute 30, 1689-1697 (2005).
[11] B. F. Kock, T. Eckhardt, and P. Roth, "In-cylinder sizing of Diesel particles by time-resolved laser-induced incandescence (TR-LII)," Proc. Combust. Inst. 29, 2775-2781 (2002).
[12] B. F. Kock, T. Eckhardt, and P. Roth, "In-cylinder sizing of diesel particles by time-resolved laser-induced incandescence (TR-LII)," Proceedings of the Combustion Institute 29, 2775-2782 (2002).
[13] R. Starke, B. Kock, and P. Roth, "Nano-particle sizing by laser-induced incandescence (LII) in a shock wave reactor," Shock Waves 12, 351-360 (2003).
[14] P. Roth and A. V. Filippov, "In situ ultrafine particle sizing by a combination of pulsed laser heatup and particle thermal emission," Journal of Aerosol Science 27, 95-104 (1996).
Temperature measurement in sooting flames
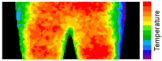
Two-Line-LIF-Thermometry
The relative population of different states (translational, rotational, vibrational and electronic) of an atom or molecule dependents on temperature. Therefore, every measurement method capable of probing the population of one or multiple states can in principle be used for temperature measurements. For the practical application, apart from the experimental realization, the probed state must have an energy that corresponds to the temperature range that should be measured. Laser-induced fluorescence (LIF) is a flexible method for probing state populations. A number of methods has been developed for temperature measurements [1]. Depending on the number of states probed, we distinguish between one-, two-, and multi-line methods. Our group is developing methods for temperature measurements in sooting and non-sooting flames using the nitric oxide (NO) molecule as temperature probe [2]. Two- as well as multi-line approaches are investigated. In the two-line approach, the populations N1 and N2 of two different vibrational states are measured using laser-induced fluorescence. The temperature dependence of the populations depends on the energy difference Δε of the states and is given by a Boltzmann expression
with k, Boltzmann constant.
The advantage of this two-line method is the independence of the local NO concentrations through the ratio in the given equation. We applied the method in lean and sooting laminar ethylene/air flames (see figures). The NO molecule is an excellent temperature probe for experimental as well as spectroscopic reasons. Due to its stability at high temperatures, it is naturally formed in most combustion systems or can be added to the flame. It has a relatively large absorption cross section, high fluorescence efficiency. Furthermore, the molecule is spectroscopically well characterized which is important for interpretation of the LIF signals.
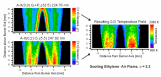
Figure 1: Temperature field in a cross-section through a sooting ethylene-air flame
References
[1] Laurendeau N. M., Temperature Measurements by Light-Scattering Methods, Prog. Energy Combust. Sci. 14, 147-170 (1988).
[2] W.G. Bessler, F. Hildenbrand, C. Schulz, Two-line laser-induced fluorescence imaging of vibrational temperatures of seeded NO, Appl. Opt. 40, 748-756 (2001).
[3] W. G. Bessler and C. Schulz, "Quantitative multi-line NO-LIF temperature imaging," Appl. Phys. B 78, 519-533 (2004).
[4] T. Lee, J. B. Jeffries, R. K. Hanson, W. G. Bessler, and C. Schulz, "Quantitative NO-LIF Temperature Imaging in High-Pressure Flames," in 41st AIAA Aerospace Sciences Meeting and Exhibit, January 6-9 (Reno, NV, 2003), Paper No. 2003-0583.
[5] T. Lee, W. G. Bessler, H. Kronemayer, C. Schulz, and J. B. Jeffries, "Quantitative temperature measurements in high-pressure flames with multi-line nitric oxide (NO)-LIF thermometry," Appl. Opt. 31, 6718-6728 (2005).
[6] H. Kronemayer, W. Bessler, and C. Schulz, "Gas-phase temperature measurements in evaporating sprays and spray flames based on NO multiline LIF," Appl. Phys. B 81, 1071-1074 (2005).
[7] H. Kronemayer, I. Düwel, and C. Schulz, "Temperature imaging in spray flames," in European Combustion Meeting (Louvain-la-Neuve, 2005).
Chemiluminescence
Most naturally emitted ultraviolet and visible radiation of flames, called chemiluminescence, is caused by short lived electronically excited intermediate species such as OH*, CH* or C2* formed during chemical reactions [1]. An example is depicted in Fig. 1 (left panel) from a Bunsen burner, operated with a stoichiometric mixture of methane and air. If the radiation emitted by this flame is dispersed in a spectrometer into its wavelength components, spectra can be observed like those shown in Fig. 1 (right panel). On recognizes characteristic emission bands originating from the above mentioned species in their respective electronically excited (A) states of OH* (310 nm), CH* (388, 431 nm) und C2* (473 nm), respectively. It is therefore of interest to investigate if the intensities or spectral shapes of these band systems can provide quantitative information on, e.g., local fuel/air ratios, heat release rate (HRR) or chemical processes of the combustion system investigated. If so, this would be of great importance for technical combustion systems, since detecting chemiluminescence radiation is a cheap and non-intrusive method for monitoring the combustion event in environments such as power plants, waste incinerators or combustion engines.
From a technical viewpoint operating flames with chemiluminescence detection is a simple and low budget method for continuous monitoring and control of large scale combustion systems, such as in power plants or combustion engines. Therefore, basic research in quantifying chemiluminescence with respect to heat release rate and fuel/air ratio measurements is of high importance. For this purpose, in our group we investigate stable, laminar counterflow diffusion as well as turbulent swirl flames at atrmospheric pressure, respectively, at a variety of operating conditions. In the first case flame stretch and fresh gas composition (fuel composition, fuel/air ratio) can be varied, whereas in the second case besides fresh gas composition the extent of swirl can be changed within certain limits. For generating the first type of flames a special burner was constructed and built as depicted in Fig. 2 (left panel). Chemiluminescence spectra for each species were recorded with the spectrometer/camera combination shown in the right panel of Fig. 2, and spatial profiles of chemiluminescence intensities were evaluated as a function of distance from the fuel supply cylinder (cf., Fig. 3).
With respect to obtaining a measure for the heat release rate in flames chemiluminescence measurements will be compared with an alternative method, i.e., the detection of hydroxyl radicals (OH) and formaldehyde (H2CO) in their electronic ground states (X). The latter will be detected using the modern laser-spectroscopic diagnostic method of laser-induced fluorescence (LIF). By this technique laser beam, whose wavelength is absorbed by the respective molecule, is formed into a thin light sheet and sent through the flame gases. Absorbing molecules distributed within the excitation volume will be excited and subsequently send out fluorescence radiation in a characteristic wavelength region, which can be analyzed for species concentration (and temperature). In the specific application the product of the two-dimensional distributions of OH and H2CO species is proportional to the local heat release rate in the common overlap region of two separate light sheets used for each species excitation.This procedure for HRR measurement is based on the fact that the most important elementary reaction responsible for heat release in premixed flames is given by:
CH2O + OH --> HCO + H2O,
and that the product of the reactant concentrations is a measure for this quantity. Experimentally, this requires the simultaneous and instantaneous capture of a relative signal intensity from both species - if possible in a two dimensional field of view [2]. Subsequently, this product of both LIF signal intensities, (ILIF, CH2O (T) × ILIF, OH (T)), is formed, which then represents the spatial region within the light sheet where the heat release takes place. Such results obtained from in-situ laser diagnostic measurements in flames are an important data base for, on the one hand the validation of the chemiluminescence measurements as well as chemical kinetics mechanisms for simulating these combustion systems.

Figure 3: spatial profile of chemiluminescence intensities of OH*, CH*, C2* und CO2*, as measured in the diffusion flames of fig. 2.
Literatur
[1] A.G.Gaydon and H.G.Wolfhard,Flames: Their Structure, Radiation,and Temperature(Chapman and Hall, London, 1978).
[2] P.H.Paul and H.N.Najm,"Planar laser-induced fluorescence imaging of flame heat release," Proc.Combust.Inst.27,43-50 (1998).
Gas-phase temperature measurements in Bunsen type flamesTemperature Measurements
The gas temperature in an atmospheric pressure ethylene/air premixed Taran burner flame was measured using the multi-line NO-LIF thermometry. The results are presented in Fig.1. Photographs are shown in the 1st row. The technique yields the temperature field (2nd row), the NO-LIF signal strength (3rd row), and the scattering background as the baseline of the spectra (4th row). In the sooting flame, the scattering off the soot particles gets so strong that a detection light filter has to be used to block the scattering and only detect NO-LIF.
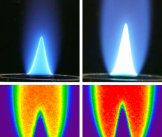
Figure 1: 1st row: Photograph of the flame; 2nd row: gas-phase temperature field; 3rd row: NO-LIF signal strength; 4th row: Scattering background as baseline of the spectra
The automated data interpretation is carried out with the spectra simulation program lifsim (www.lifsim.com) that has been developed in house.
Visualization of gaseous flows and mixing processes via „Tracer"-LIFTracer LIF
In combustion and other processes it is often desirable to experimentally visualize the mixing of gaseous flows. In gas turbines, chemical reactors, internal combustion engines, fuel cells etc., this process often happens under unknown environmental conditions of temperature, pressure, and gas composition. In the case that species within the mixture fluoresce upon excitation with a UV laser, laser-induced fluorescence, LIF, can be used to locally measure the species concentration in the flow - often in imaging configurations that yield two-dimensional concentration maps.
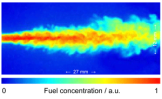
Figure 1: Turbulent mixing of gaseous flows, visualized with tracer LIF
However, in many cases such species are not naturally part of the gas mixture. In these cases, a suitable „tracer" is mixed to the respective gas component. This tracer should exhibit an evaporation behavior similar to the respective gas component (e.g., the fuel), and its absorption and fluorescence behavior should be well known to enable quantitative interpretation of LIF signal intensities. Typical tracer molecules for this kind of optical diagnostics are ketones (acetone, 3-pentanone) or aromatics (toluene, naphthalene, etc.). As an example figure 2 (left) shows the fluorescence spectrum of toluene after excitation with 266 nm from fourth harmonic of a Nd:YAG laser [1,2].
For extracting quantitative information out of measured LIF signal intensities (e.g., temperature or concentration distribution of fuel vapor with tracer additive in the probe volume) the photo-physical properties of the tracer must be known. This includes the dependence of the absorption spectra and the fluorescence quantum yield on temperature and pressure [3].

Figure 2 left panel: Emission spectra of toluene vapor after excitation with 248 nm (from a KrF excimer laser) at different temperatures.

Right panel: high temperature, high pressure flow cell for investigating tracer-seeded gaseous mixtures at temperatures up to 1200 K and pressures up to 10 bar, respectively. The cell has for optical windows for introducing laser beams and detection of fluorescence radiation.
At IVG an optically accessible high-pressure high-temperature cell is available for the systematic study of tracer-seeded gas flows in the pressure and temperature range between 1 - 10 bar and 300 - 1400 K. To avoid pyrolysis of the tracers at high temperatures the gas mixtures are constantly exchanged in a flow configuration. Figure 2 (right panel) shows the cell, the gas supply, and the electrical control systems. The gaseous flows (tracers liquid at room temperature are evaporated before mixed with other gases) are metered by electronic flow controllers.
Tracers are excited in the near UV spectral region by a Nd:YAG laser at 266 nm and fluorescence spectra are recorded using a combination of a spectrometer and a CCD-camera (see Fig. 2, right panel).
The left panel in Fig. 3 presents the pressure dependence of the fluorescence intensity of 3-pentanone when this tracer is mixed in three different carrier gases (nitrogen, air and oxygen, respectively). The right panel in Fig. 3 shows the behavior of the fluorescence signal intensity of toluene as a function of air pressure. One notices the strong decrease of the signal intensity with increasing air pressure due to the efficient collisional quenching of fluorescence radiation by the increasing concentration of molecular oxygen.
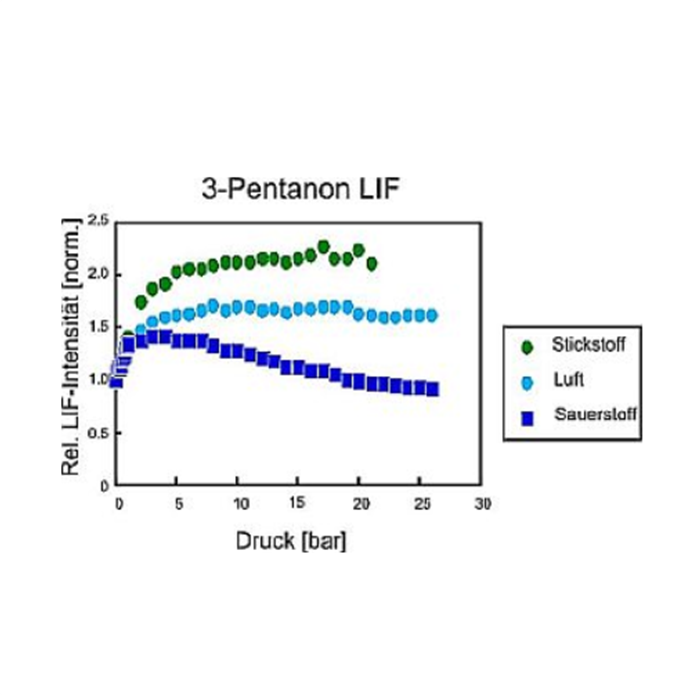
Figure 3 Left panel: dependence of measured LIF signal intensity of 3-pentanone on gas pressure in three different carrier gases (N2, air, O2, respectively).

Right panel: dependence of LIF signal intensity of toluene as a function of total air pressure [1].
References
[1] W. Koban, J. D. Koch, R. K. Hanson, and C. Schulz, "Oxygen quenching of toluene fluorescence at elevated temperatures," Appl. Phys. B 80, 777-784 (2005).
[2] W. Koban, J. D. Koch, R. K. Hanson, and C. Schulz, "Absorption and Fluorescence of Toluene Vapor at Elevated Temperatures," Phys. Chem. Chem. Phys. 6, 2940-2945 (2004).
[3] W. Koban, J. D. Koch, V. Sick, N. Wermuth, R. K. Hanson, and C. Schulz, "Predicting LIF signal strength for toluene and 3-pentanone under engine-related temperature and pressure conditions," Proc. Combust. Inst. 30, 1545-1553 (2005).